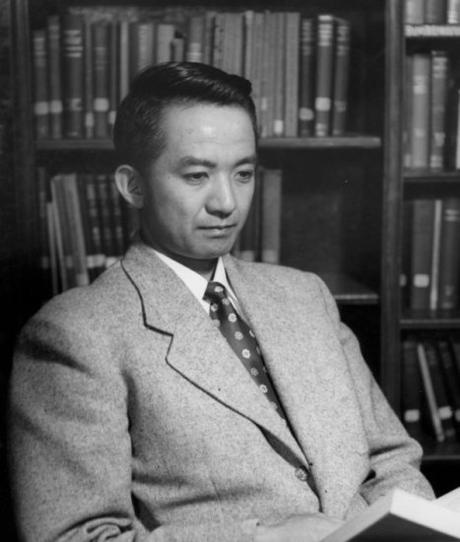
By Dr. Marcus Calkins, Part 2 of 3
The molecular defect in Sickle cell disease was demonstrated by Linus Pauling’s lab in 1949, and one year prior, James Watson had proposed that increasing the level of fetal hemoglobin may provide a means by which the disease could be cured. These two publications provided a direction for what may soon become a real-life cure for the disease. However, many practical questions needed to be answered before a cure could be realized, or even imagined in a realistic sense. The first of these questions had to do with the composition of hemoglobin protein.
Defining the structure of hemoglobin (1950s-1960s)
The project in the Pauling lab was led by a formidable graduate student of Japanese descent named Harvey Itano. It is noteworthy that despite his birth in Sacramento and his honorific as valedictorian of the University of California, Berkeley class of 1942, Itano was interned at Tule Lake during World War II. He was only released from detention to attend medical school at Washington University in St. Louis. Upon graduating with his M.D., he came to Pauling’s lab to pursue a Ph.D. His widely celebrated doctoral thesis was built on the Sickle cell disease project. After finishing this training in biochemistry and publishing his high-impact paper with Pauling, Itano opened his own lab in which he continued working on hemoglobin.
By applying many of the same methods pioneered in Pauling’s lab, Itano’s small group became central players in identifying aberrant forms of hemoglobin that lead to disease. Because of this careful and detailed work, Itano and other scientists began to suspect that the protein subunits that make up hemoglobin may be derived from multiple, highly similar genes. At the time, this idea represented a major paradigm shift, as it was previously assumed that each enzyme should correspond to a single gene.
This idea also brought up an evolutionary question of how such similar genes might come to exist. In a memorial biography, Itano’s friend and colleague R.F. Doolittle summarized Itano’s forward thinking on the genetic and evolutionary basis of enzyme constituents as follows:
In his thorough review of all the data, Harvey noted that in sheep and cattle, however, there were two kinds of end group (valine and methionine), and concluded that there had to be two each of two kinds of polypeptide in human adult hemoglobins. He went on to discuss how gene duplications could account for all the various hemoglobin chains, including myoglobin and foetal hemoglobin. These were thoughts well ahead of their time.
These major scientific contributions and others led to Itano’s election to the National Academy of Sciences, U.S.A. He was the first of many Japanese Americans to achieve that honor.
The exact change in hemoglobin protein responsible for causing Sickle cell disease was identified at the amino acid level ten years after Itano’s paper with Pauling, when V.M. Ingram used protease digestion to show that the disease came from a glutamine-to-valine mutation in the β-globin subunit of the hemoglobin protein complex. Although the molecular structure of DNA was accurately modeled by Watson and Crick in 1954, revealing tantalizing clues as to how genetic information can be encoded and passed from cell to cell and parent to child, the precise genetic mutation underlying Sickle cell disease would remain unknown for a few more decades, until the molecular biology revolution.
Molecular Switch from Fetal to Adult Hemoglobin (1970s-1990s)
In order to institute the cure dreamed up by Watson, much needed to be learned about hemoglobin composition, genetics, and control in red blood cells. This basic knowledge was largely generated through new technologies developed in the latter part of the 20th century. The cell and molecular biology revolution began in the mid-1970s and brought with it new techniques for manipulating DNA and other molecules. These advances allowed scientists to define genes and genetic structures, and to begin to understand how gene expression is regulated.
With regard to hemoglobin, it was discovered that two sets of genes (five on Chromosome 11 and three on Chromosome 16) encode the subunit proteins for the hemoglobin molecular complex. Each set contributes one subunit to the complex, and the subunits that are present in the blood change, depending on the developmental stage of the individual. In essence, a switch occurs just after the time of birth, wherein expression of fetal hemoglobin is turned off and adult hemoglobin is turned on. The mechanics of this switch, including the DNA sequences and proteins that make it work, were elucidated during this fruitful period of biological research.
Once the mechanics of the fetal hemoglobin to adult hemoglobin molecular switch were known, it became apparent that one gene, BCL11A, is a dominant factor in shutting down fetal hemoglobin production. If this gene can be silenced or disrupted, fetal hemoglobin expression will continue. Furthermore, there is strong evidence from molecular, cellular and clinical studies that continued expression of fetal hemoglobin will at least partially prevent the harmful accumulation of mutant hemoglobin aggregates and thereby prevent Sickle cell disease.
However, turning off the BCL11A gene is not an easy task. The molecular system evolved over millions of years to function robustly under almost any environmental condition or situation. Like a train hurtling down a track, such developmental programs are extremely hard to redirect, and if they are completely derailed, the individual may experience catastrophic effects. Thus, precise control of only the BCL11A gene, precisely in red blood cells, is necessary to realize the therapy first imagined by Watson. This level of control is possible to achieve in cells outside the body, but inside the body, cells are resistant to change, and our ability to target only one cell type at a time remains relatively primitive. Thus, a cure for Sickle cell disease still needed a method for regulating BCL11A specifically in red blood cells.